A Better Look
The development of cryo-EM has revolutionized structural biology
- 12 minute read
- Feature
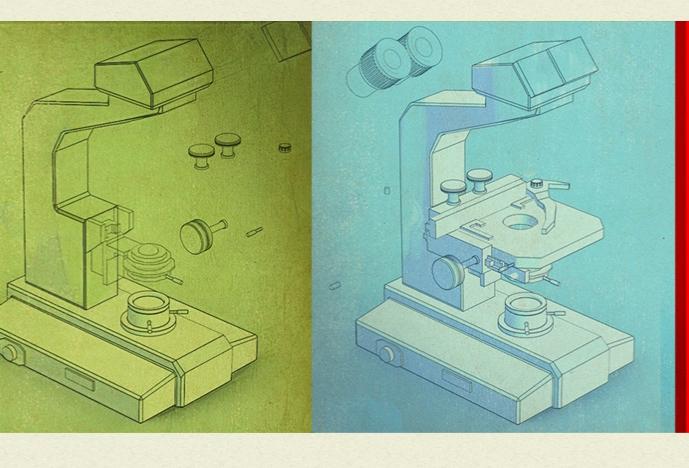
Eric Fischer hadn’t intended to help figure out the biological mechanism of one of the more infamous drugs in history. Nor had he envisioned playing a role in sparking a new field of drug development. He had just wanted to see what thalidomide looked like in action at the atomic level. He was, after all, training to become a structural biologist.
“We looked at it out of pure curiosity,” recalls Fischer, now an HMS assistant professor of biological chemistry and molecular pharmacology at Dana-Farber Cancer Institute. “It was fascinating that there was so little known about its mechanism of action.”
When thalidomide hit German pharmacies in 1957, the over-the-counter sedative became an instant blockbuster. Marketed as a wonder cure for insomnia and morning sickness, it was said to be so safe that scientists “could not find a dose high enough to kill a rat.” Soon, it was being distributed in forty-six countries, often to pregnant women.
Then, wonder turned to horror: As a result of in utero exposure to the drug, more than 10,000 children in Germany alone were born with devastating birth defects. By the early 1960s, thalidomide was being frantically pulled off the world market.
This tragedy raised many scientific questions. How did thalidomide cause the malformation of arms, legs, and organs during fetal development? How did the drug, as clinical trials later revealed, slow the growth of cancers such as multiple myeloma, for which the drug is now a frontline treatment? The answers eluded scientists for decades.
An important clue came in 2010 when researchers discovered that thalidomide binds to a component of a certain form of ubiquitin ligase that cells use to tag proteins for destruction. For Fischer, this discovery proved timely. He happened to be studying the molecular structure of ubiquitin ligase using the workhorse of structural biology, x-ray crystallography.
Fischer and his colleagues painstakingly purified and crystallized the specific ubiquitin ligase bound with thalidomide, and then blasted it with x-rays. They used the pattern that formed as the x-rays passed through the crystal to deduce the arrangement of its atoms—the same technique Rosalind Franklin employed in the 1950s to generate the data used to solve the structure of DNA.
After some data crunching, the researchers saw something interesting: a nearly imperceptible handful of atoms nestled in a tiny pocket on an enormous protein complex.
They had located the thalidomide.
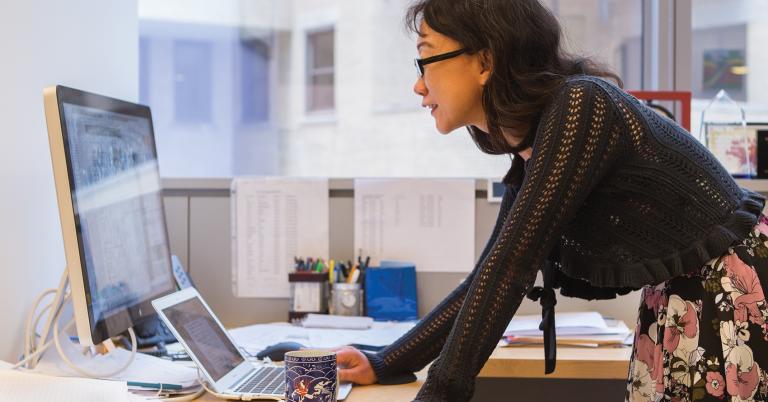
Like a speck of dust in the delicate gears of a watch, thalidomide’s presence was enough to perturb the function of the ubiquitin ligase. One of the complex’s normal targets could no longer bind in that pocket. Instead, thalidomide caused the ubiquitin ligase to selectively bind, and mark for destruction, other proteins, specifically a set of transcription factors known to play a role in multiple myeloma.
As the master regulators of a cell’s biology, transcription factors play central roles in almost every disease imaginable, from cancer to cardiovascular disease to Alzheimer’s. But, says Fischer, they were considered undruggable.
“The idea of selectively degrading transcription factors has been around for a long time, but no one could ever get it to work,” Fischer says. “An understanding of thalidomide’s mechanism has shown that this is in fact very much possible, and by manipulating this mechanism, we could potentially develop new drug candidates.
“I don’t think there’s a big pharmaceutical company out there that’s not exploring this opportunity.”
Throughout history, observations of structure, from Hooke’s cells to the beaks of Darwin’s finches, have provided insights necessary to understand how life works. This is particularly true in structural biology, a discipline focused on visualizing life at its most fundamental. Discoveries of the atomic structures of important proteins and biological molecules have been among the most celebrated in science and have generated more than a dozen Nobel Prizes, new fields of research, and multibillion-dollar companies.
Despite their successes, structural biologists have struggled to keep pace as technology transforms the study of biology. Every day, researchers reveal more of the molecular processes that underpin life, and biopharma companies, racing to manipulate newly discovered biological targets, pop up like mushrooms. The tools that structural biologists have relied upon were developed decades ago, so the functional mechanisms of these myriad new molecules often remained invisible.
How many other thalidomides wait to be seen?
So, when researchers began publishing near-atomic resolution structures of complex proteins using a technique known as cryo-electron microscopy (cryo-EM) in the early 2010s, structural biologists around the world declared, without hyperbole, that a revolution had begun.
Ahead of its time
In 2015, the journal Nature Methods named cryo-EM, which involves flash freezing molecules and imaging them with an electron microscope to deduce their structure, the Method of the Year. In 2017, cryo-EM pioneers received the Nobel Prize in Chemistry. National Institutes of Health director Francis Collins has blogged about its implications for drug discovery. To many, scientists and nonscientists alike, the technology seemed to emerge from nowhere. Yet structural biologists knew it had been decades in the making.
“The triumph of twentieth-century biology was discovering that cells speak the language of chemistry and that the structure and interaction of molecules determine the phenomena that we call biology,” says structural biologist Stephen Harrison, PhD ’68, the HMS Giovanni Armenise-Harvard Professor of Basic Biomedical Science.
“Cryo-EM is critical to how we will translate those lessons into knowledge that broadens our understanding of biological processes and helps us solve important problems in medicine in the decades to come,” adds Harrison, who is the director of the Harvard Cryo-EM Center for Structural Biology, a new facility jointly established by HMS, Harvard’s Office of the Provost, Boston Children’s Hospital, Dana-Farber Cancer Institute, and Massachusetts General Hospital.
Theory and practice
Despite being one of the most productive scientific tools in history, x-ray crystallography has limitations. Coaxing a protein to form a large, perfectly aligned crystal—like a brick made up of the same repeating component part—is unpredictable and can take years of empirical testing to achieve.
Many proteins, either because of their complexity or composition, simply cannot be crystallized. Even when the process is successful, crystal structures fix a protein in an unnatural state and offer little insight into the protein’s different conformations and interactions.
It was his frustration with x-ray crystallography that drove structural biologist Richard Henderson, who shared the 2017 Nobel Prize in Chemistry for his contributions to cryo-EM, to seek alternatives in the 1970s.
“The specimen we had wasn’t working with x-rays, so we thought we’d try something else,” says Henderson, who is based at the MRC Laboratory of Molecular Biology in Cambridge, England.
Henderson turned to electron microscopes, which fire a beam of electrons that interact with the atoms of the sample they pass through. These interactions are picked up by an electron detector and used to create a highly magnified image of the sample.
In 1975, Henderson and his colleague, Nigel Unwin, became the first to use an electron microscope to derive the 3D structure of a protein crystal. Although the work established proof of principle, the resolution remained far from that achieved through x-ray crystallography. Still, Henderson and others pushed forward.
In the early 1980s, Henderson’s fellow 2017 Nobel laureate, Jacques Dubochet, now at the University of Lausanne in Switzerland, put the “cryo” in cryo-EM when he developed a way to flash freeze a sample of a purified protein in solution. This process preserved the natural atomic state of the protein and held the molecules nearly motionless so they could be imaged. Scientists suddenly did not need to crystallize samples to see individual isolated proteins.
Yet, without crystallization, a protein of interest orients randomly throughout a solution, producing a confusing mess that is difficult to analyze.
Fortunately, the third of the 2017 Nobel laureates, Joachim Frank, currently at Columbia University in New York, was already working on a solution. He was developing sorting algorithms that could process images of thousands of individual, randomly oriented molecules and group them by shape. By averaging similarly shaped groups together, Frank could reconstruct 2D images of a protein from essentially every point of view. These 2D images could then be composited into a 3D model of the protein being studied.
By the 1980s these innovative approaches had effectively created cryo-EM as it exists today. It took decades, however, before it became clear whether cryo-EM would ever be a broadly useful technology. A key moment came in 1995, when Henderson calculated and published a seminal review outlining what was needed to achieve atomic resolution with cryo-EM.
“From then on our goal was to realize what the theory suggested we should have,” says Henderson.
“So, for 20 years, we’ve known that cryo-EM was going to work,” he adds. “It just needed technical problems to be solved.”
They also had to wait for the smartphone to be invented.
Camera work
Like many researchers, Hao Wu, the HMS Asa and Patricia Springer Professor of Structural Biology at Boston Children’s Hospital, turned to cryo-EM out of necessity. Her lab studies signaling proteins, particularly ones that cells use to coordinate the body’s innate immune response. These proteins are essentially alarm bells, recruiting immune cells and promoting inflammation when a foreign invader is detected. Understanding their structure and functional mechanisms is critical, Wu says, since inflammation not only can be damaging but is also increasingly being linked to diseases from cancer to neurodegeneration.
A few years ago, when cryo-EM was still being derided as “blobology” because of the low-resolution, blob-like structures it produced, Wu discovered that the signaling proteins she was studying formed complexes that could not be crystallized. The complexes were also too large to be analyzed using the other primary structural biology tool, nuclear magnetic resonance spectroscopy. To gain the structural insights needed to develop new therapeutic strategies against the diseases in which these proteins may be involved, Wu needed an alternative.
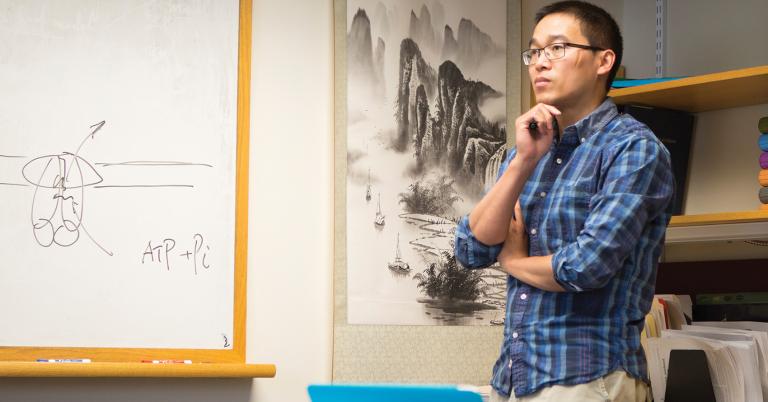
“Our move to cryo-EM was driven by biology, not technology,” Wu says. “I think we were in the right place at the right time—suddenly cryo-EM became so much better.”
That improvement spun from a new generation of image sensors developed in response to consumer demand for better cell phone cameras. A number of key players, including Henderson, worked with manufacturers to leverage new sensor technology into greatly improved direct electron detectors for cryo-EM. These new detectors could even take movies. Movies allowed for the computational rectification of movement, induced by the energy of the electron beam, that occurred in a sample. The result: blur-corrected images.
Much better
Combined with dramatic increases in computational power and improved algorithms, cryo-EM specialists could now generate hundreds of thousands, even millions, of blur-corrected images of a protein and use them to calculate 3D atomic models relatively quickly. Formerly blurry models soon revealed functionally important side chains. The resolution revolution had begun.
“When you know which atom is next to which atom, it’s almost like molecular Lego for drug discovery,” says Wu. “You can look at how the atoms fit, calculate how they’re binding, and design better-targeted molecules.”
More than looks
During his postdoctoral research, structural biologist Maofu Liao had to choose: specialize in tried-and-true x-ray crystallography or in the upstart, cryo-EM.
Liao chose cryo-EM.
“We think about proteins as molecular machines because in their native environment different parts move and do so repeatedly,” says Liao, now an HMS assistant professor of cell biology. “But until cryo-EM, we mostly saw the details of proteins only in a fixed shape and conformation.”
“We’re not happy anymore with just seeing what a protein looks like,” he adds.
Liao has been at the forefront of efforts to reveal the structures of dynamic proteins embedded in cell membranes, which are extraordinarily difficult to study with x-ray crystallography. These proteins exist in an ever-shifting sea of lipids—many are in constant motion; opening and closing during chemical transport; spinning like motors to generate energy, even, as in the case of the lipopolysaccharide flippase in bacteria, moving a large lipid molecule attached with chains of sugar to build protective walls around cells.
Using cryo-EM, however, Liao and colleagues have revealed exactly how these and other proteins carry out such remarkable feats.
“People have long said that structure is function,” says Liao. “I think that was a more conceptual statement in the past, but will become more realistic in the coming years. I’m very optimistic about the future of cryo-EM, not because it will help us discover more new structures, but because it will allow us to directly visualize function.”
The addition of cryo-EM to the structural biology toolbox has essentially opened a door to a new world of biological insight. But cryo-EM development is far from finished. An emerging technique known as cryo-electron tomography, something like an atomic-level CT scan, could soon allow researchers to study proteins in the cellular environment and not solely as isolated molecules in solution.
“It’s clear that the field is headed toward studying the structure, organization, and interaction of molecules as they exist in their native environment, for example, determining the structures of ribosomes as they make a protein in a cell,” Harrison says.
“But the key to structural biology,” he adds, “has always been great biochemistry and sample preparation: Otherwise, you’re imaging something meaningless. Many labs interested in structure are going to have to first relearn how to be biochemists.”
Ever the visionary, Henderson, who knew decades ago that the promise of cryo-EM was just a technical issue or two from being realized, sees a bright future ahead.
“Cryo-EM is already the dominant technology in structural biology,” Henderson says. “The goal now is to make it cheaper, faster, and more reliable, so that anybody can use it. I think it will someday become a common technique like measuring pH or sequencing DNA. It will only get more dominant.”
Kevin Jiang is a science writer in the HMS Office of Communications and External Relations.
Images: Tavis Coburn (top illustration); John Soares (Wu and Liao)